TeraHertz λ-Photonics
Enhancing THz field mater interactions down to the nanoscale
THz spectroscopy is a powerful tool for studying materials, but biological objects often pose numerous challenges. Due to their small size, typically below the wavelength of THz radiation (ranging from tens of micrometers to millimeters), biological objects including nano-sized proteins, viruses, bacteria and even, the standard sample use for protein study, the protein micro crystal, interact weakly with THz beams, making them difficult to measure. This explains the limited success in obtaining relevant THz spectra for biological macromolecules until recently.
Researchers have developed several strategies to overcome this challenge, including near-field microscopy. In our group, we are exploring the use of integrated photonics. This approach utilizes devices where light is confined in modes which size can be as small as the wavelength divided by the highest refractive index used in the design. A key advantage of the THz range is the relatively low metal loss compared to higher frequencies. This allows us to design devices with metallic structures, drawing inspiration from established designs used in the microwave and radar spectral range (sometimes dating back a century).
We follow two approaches: broadband and resonant.
The broadband approach, depicted on fig 2, utilizes subwavelength thick-slot-waveguides. These waveguides offer several advantages for studying biological samples with THz spectroscopy. They confine the light in a mode as small as the subwavelength gap between the two metallic sides, enabling efficient interaction with even tiny biological objects. We are using its first order mode that is quasi TEM and has very low losses and dispersion. Additionally, they possess an open guiding section where samples can be placed, allowing direct interaction with the confined light mode. Furthermore, these waveguides benefit from planar fabrication processes, making them compatible with microfluidic integration for future bioanalysis applications.
To couple the incoming propagative wave to the wave-guide, we coupled it to a tapered planar antenna leading to this shape giving it the name of the butterfly. The butterfly device can achieve field enhancement by a factor of 100 to 1000, depending on the waveguide width. This significant enhancement played a crucial role in enabling us to measure the broadband spectrum of a miniscule sample (1 nL) of dried glutamic acid molecular crystal up to 3 THz.
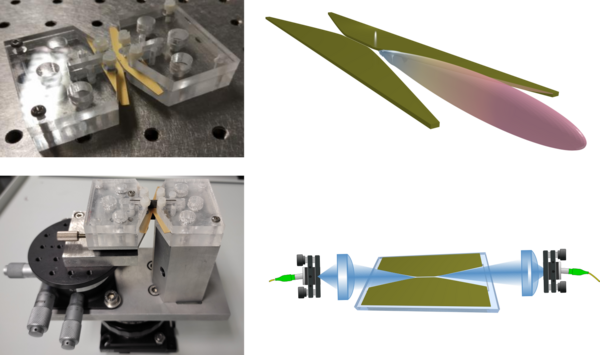
The resonant approach offers a complementary strategy not only confining light in space but recycling it in time. It is inspired by resonant terahertz metasurfaces, which are patterned metallic structures enhancing light-matter interactions. These surfaces are composed of repetitive building blocks called meta-atoms. In our research, we use a specific type of meta-atom called a split ring resonator (refer to Figure 3). This resonator can be visualized as an LC circuit, with the loop acting as the inductance and the gap functioning as the capacitance. The electric field of the resonant mode is strongly confined in a volume less than λ3 within the gap of the split ring resonator, leading to significant field enhancement. This enhanced field makes the resonant approach highly sensitive to any material placed within the gap.
A key challenge in using these metasurfaces for biological samples is ensuring a consistent sample positioning across all the meta-atoms. To address this, we have significantly improved our Time-Domain Spectroscopy (TDS) experiments. We essentially transformed them into a true microscope with a focal spot size of (λ/2)×(f/D). We have also developed a comprehensive methodology to improve the signal-to-noise ratio and accurately estimate uncertainties in our experiments. This combination allows us to measure single meta-atoms in a reproducible manner over a broad frequency range (more than a decade). Furthermore, the high field enhancement (estimated at 10,000 to 100,000) enabled us to perform narrowband spectroscopy of glutamic acid on a sample as small as 15 fL– 10⁻¹³ mol.
These two approaches, broadband and resonant, lay the foundation for further advancements. Our future work will explore variations on these themes, such as microfluidic integration and cryogenic operation. The ultimate goal is to improve even more the light-matter interactions at subwavelength scales and achieve strong coupling, paving the way for exciting new experiments not only probing the macromolecule but also acting on them.